Metal-catalysed Reduction
Mechanism + Description
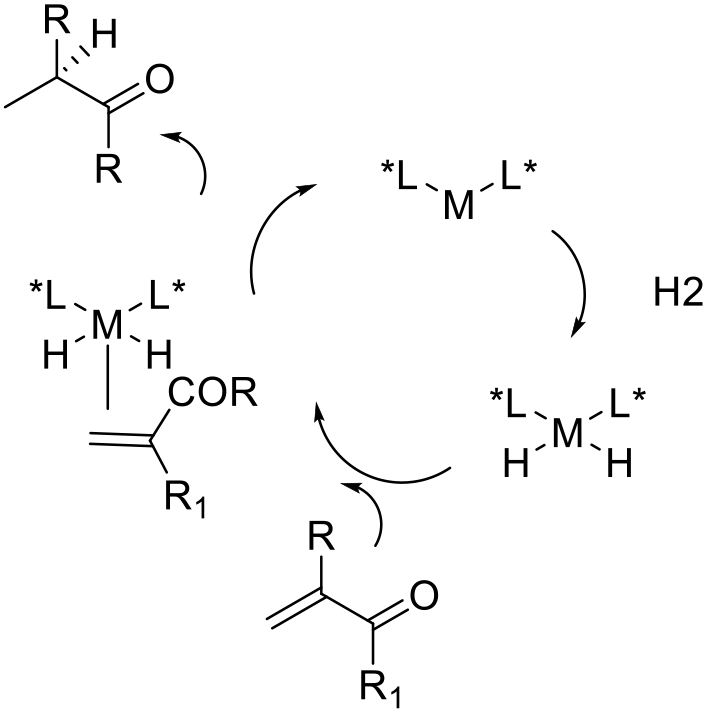
The mechanism of asymmetric reduction can be complex and dependant on metal, the charge on the active catalytic complex, substrate, and other factors like hydrogen pressure. The key steps are generation of the catalytically active complex, oxidative addition of H2, and coordination of the ketone. The chiral induction in the product is kinetically determined, and governed by the geometry of the catalyst installed by the chiral ligands.
General comments
While homogeneous metal complexes are rarely used for the racemic reduction of ketones to chiral alcohols, the use of homogeneous metal complexes with chiral ligands to produce chiral alcohol products has become a very common and scalable transformation. Metal catalysts can be added as preformed complexes or formed in situ from ligands and a metal source. Typically Rh, Ir and Ru metals are employed, but in common with other transformations catalysed by precious metals, there is a great deal of interest in developing analogues reactions using chiral complexes containing base metals like Fe, Co , Ni.
Metal complexes are available that allow selective reduction of ketones to chiral alcohols in the presence of other reducible functionalities such as alkenes.
The reductant is either hydrogen gas, or nascent hydrogen from transfer hydrogenation donors, typically formic acid or Et3N HCO2H mixtures. Typically ligand-metal combinations are developed for H2 or transfer hydrogenation reduction, and may not be transferable between the hydrogen sources.
Key references
Relevant scale up examples
Org. Proc. Res. Dev. 2013, 17, 307−312.
13 kg scale
Org. Proc. Res. Dev. 2011, 15, 1178–1184.
40 kg scale
Org. Proc. Res. Dev. 2016, 20, 81−85.
30 kg scale
Org. Proc. Res. Dev. 2015, 19, 315−319.
7 kg scale
Org. Proc. Res. Dev. 2007, 11, 519-523.
140 kg scale
Org. Proc. Res. Dev. 2015, 19, 315−319.
7 kg scale
Org. Proc. Res. Dev. 2007, 11, 519-523.
140 kg scale
Org. Proc. Res. Dev. 2020, 24, 1164–1174
660 kg scale
Green Review
-
Atom efficiency (by-products, molecular weight)
Reduction with stoichiometric boron reagents has very poor atom economy. Addition of two hydrogens to the substrates generates by-products of 354 molecular weight. Generally such reactions also consume very large volumes of solvent in removing these by-products and are thus very mass inefficient.
Reduction with catalytic boron complexes are much better, however the catalyst stoichiometry needs to be optimized.
With optimized metal and ligand stoichiometry, catalytic metals and ligands have negligible contribution to the atom/mass intensity. Hydrogen is typically used in large molar excess, but this is non-polluting.
Biocatalysis is an efficient catalytic technology, but enzyme loading should be optimized and steps using stoichiometric reagents, like cofactor recycle, should be optimized to avoid unnecessary excess of reagents. - Safety Concerns
No major concerns around scaling asymmetric hydrogenation reactions beyond those for handling hydrogen at pressure. Generally, the lowest pressure for acceptable performance is recommended for safety, but this can be at the expense of the S/C ratio—many catalysts performing better ( TON & TOF) at higher H2 pressure (although a few give better ee at lower H2 pressure). Exotherms maybe experienced when hydrogen is introduced to the reactor.
No real concerns—enzymes are non-toxic and readily biodegradable. If live GMO cells are use, these need to be passivated before discharge into the environment. - Toxicity and environmental/aquatic impact
Main concern is around loss of precious metal/ heavy metal catalysts into waste streams. Most precious and heavy metal levels are tightly regulated. The same applies to potential metal carry through into the API. See later slide for more details on permitted. Hydrophobic, high mol. weight phosphines and phosphine oxides can be persistent and bioaccumulative and should not be discharged into aqueous waste streams.
Relevant regulatory guidance on permitted metal levels in API’s should be consulted.
For biocatalysis, there are no real concerns—enzymes are non-toxic and readily biodegradable. If viable GMO cells are use, these need to be passivated before discharge into the environment.
There may be issues with discharging aqueous waste with high boron content. Emerging data suggest boron compounds maybe more ecotoxic then previously thought. The compounds and by-products from stochiometric boron reagents are persistent and can accumulate in the environment. - Cost, availability & sustainable feedstocks
With high catalytic efficiency, this methodology can be an economical way to access chiral molecules. Precious metals like Ru, Ir and Rh are commodities and thus the price and availability can fluctuate widely with demand and general global economic cycles. Base metals are cheaper and much more abundant.
Enzymes are produced by fermentation from natural sustainable feedstocks - Sustainable implications
All metals have a high LCI impact from mining and refining operations, so use should be catalytic with efficient recovery and recycle. Ru, Ir and Rh are the most commonly used precious metal for chiral hydrogenation and these are rated at high risk of depletion. No concern for abundant base metals like Co, Ni, Fe, etc.
Enzymes are made via fermentation and cloned and recombinant enzymes would be at no risk from depletion. Enzymes break down in the environment and the constituent amino acids which are recycled in nature. The use of over expressed recombinant enzymes is generally much better than natural enzymes on a life cycle impact basis.